Limits of Synbio for Organic Chemical Production
The production of organic chemicals like methanol, ethylene, propylene (and we’ll include hydrogen and ammonia in the list, too) accounts for something like 5% of global emissions. That’s of similar magnitude to aluminum production (3%), shipping and aviation (7%), cement (10%) and steel (11%). While decarbonizing any of these sectors will be hard, organic chemicals pose a special challenge because they’re really a spectrum of products made with myriad processes.
We recently examined how different organic chemicals are manufactured today and what this implies about strategies to decarbonize if we extrapolate the near future from the present. Our findings are out in Industrial and Engineering Chemistry Research, and Ian’s note Checking our Prejudices discusses some highlights. Here, we recap and add some additional details.
Making and Decarbonizing Chemicals
Emissions from chemicals production come from two main sources: the process emissions from the energy and reactions to manufacture chemicals and the embodied emissions that get released when a chemical decomposes or is burned at the end of its life. To decarbonize chemicals, we will have to address both sources.
The chemicals made in the highest volumes—and therefore responsible for the bulk of emissions—are made using thermochemical techniques. These techniques push reactions far out of equilibrium, injecting large amounts of energy at whatever pressure and temperature is needed to maximize productivity. These reactions are carried out at awe-inducing scale. The pumps of a typical ethylene cracker are more powerful than a Saturn V rocket. Unfortunately, this productivity comes at the price of high process emissions.
In terms of embodied emissions, today’s chemicals are beholden to fossil carbons because they are easily transported and fractionated into feedstocks, carry low-cost embodied energy with which to fuel reactions, and they are inexpensive. Or one might say the environmental costs are externalized.
On the other hand, there are molecules that are hard to contemplate making through entirely synthetic means—the intricate structures of proteins or complex drugs, for example. Some molecules such as itaconic acid, didn’t become commercially available until a fermentative production route was developed, even though their chemical synthesis was established earlier. Biological synthesis can build stunning complexity in molecules, despite, or perhaps because reactions are confined to temperatures, pressures, and chemical potentials relevant to living organisms.
To decarbonize chemicals, there are two general schools of thought: (1) Use plants to get carbon from photosynthesis and convert the plants into chemicals using the tools of synthetic biology or (2) get CO2 from carbon capture processes and reduce and upgrade it through chemical means. As life rarely divides into neat binaries, there’s also the crossover case of using thermochemistry to convert biological feedstocks (think biodiesel from hydrotreated vegetable oil).[1] We’ll lump thermochemistry using agricultural feedstocks in with bioproduction because of the similarities that arise when using complex bio-feedstocks.
These two main strategies address embodied and process emissions differently. To tackle embodied emissions, the choices are to use biological feedstocks from agriculture, agricultural residues, or other biomass wastes (💩), or to get CO2 from carbon capture processes. None of these approaches are bullet-proof. Purpose-grown agricultural feedstocks come with significant ecological impacts and emissions from land use changes and synthetic fertilizers. Wastes and residues are often harder to convert than conventional feedstocks because they come from distributed sources and are more heterogeneous. As a living organism, would you rather eat the delicious glucose in a ripe grape or the recalcitrant lignocellulose of the leaves and vine? There’s a reason agricultural residues are residues. And finally, reducing CO2 into hydrocarbons will take a lot of decarbonized energy and hydrogen, posing a nontrivial infrastructure challenge.
On the process emissions side, there are also differences between chemistry and biology. Thermochemical processes tend toward higher productivity and higher process emissions, whereas biological processes such as fermentative or enzymatic reactions can have lower process emissions albeit with the downside of lower productivity. It takes energy to grow and maintain cells or produce complex enzymes, and in many biological processes, cells can be poisoned by the chemical product if its concentration is too high.
Which technique, thermochemistry or synthetic biology, is better to use for which molecules? And what might this tell us about decarbonization in the chemicals industry?
The Role of Molecular Complexity
Looking at how chemicals are manufactured today and their prices can give us a clue about when the tradeoffs above favor thermochemical vs. bioproduction processes and feedstocks. We had a hunch that complexity was a factor in when biosynthesis is used vs. thermochemistry so we mapped the manufacturing methods and prices of the top ~70 organic chemicals using a quantitative metric for molecular complexity.[2] This metric, developed by Thomas Böttcher, packages all the factors we intuitively think of as adding to complexity, (heteroatoms, number of atoms, chirality, etc.), into a convenient number. [3]
In the plot below, the categories shown as different colors are thermochemistry with traditional petrochemical feedstocks (Thermochemical-Petro), and the bioprocess categories of Fermentation, thermochemistry with agricultural feedstocks (Thermochemical-Ag), and Hybrid. Hybrid process have multiple steps, some of which are fermentative and some of which are thermochemical, for example, the conversion of fermentation products ethanol and lactic acid into ethyl lactate. The size of markers are scaled by the annual global production volumes of the products.
The plot (Figure 1) confirms our general intuition that traditional thermochemistry is favored for making low complexity molecules and bioprocesses are more prevalent at higher complexities. The transition between methods occurs at a Böttcher complexity, Cm, of around 60 (near compounds like ethyl acetate and phthalic anhydride). Price and production volume also roughly correlate with complexity.[4] For the most part, it looks like the cost of bioproducts is above ~$1000/t. So for complex products that are valuable enough, the advantages of bioprocessing tend to win out. But the simplest, cheapest, highest volume commodities require the throughput advantages of thermochemistry.
A few exceptions occur. Some exceptions indicate potential for innovation. Malonic acid has a very high price for its complexity in part because it has a low decomposition temperature making it difficult to synthesize without toxic chemicals. Perhaps there is a better way out there!
Other exceptions might indicate that molecular complexity doesn’t tell the whole story, especially for bioproducts. Ethanol, not pictured in Figure 1, is a low price and low complexity fermentation product (Cm ~ 21, price ~$550/t), as are glycerol and 1,3-propanediol. These three are products or byproducts of the biofuels push and have received significant subsidies to scaling up. Additionally, it’s not always the case that complex molecules are valuable. Sorbitol and glucose have relatively high complexities but low price. These monomeric sugars/sugar derivatives are complex, but they can be made from their biopolymer feedstocks in a few simple steps. Bio feedstocks like cellulose and starch are complex macromolecules with many chiral centers, but they are cheap because they are direct products of agriculture, requiring zero chemical conversion steps outside of farming. Likewise, biological pathways can convert complex feedstocks into specific endpoints like ethanol in a single process step, though arbitrary targets may be much more difficult.
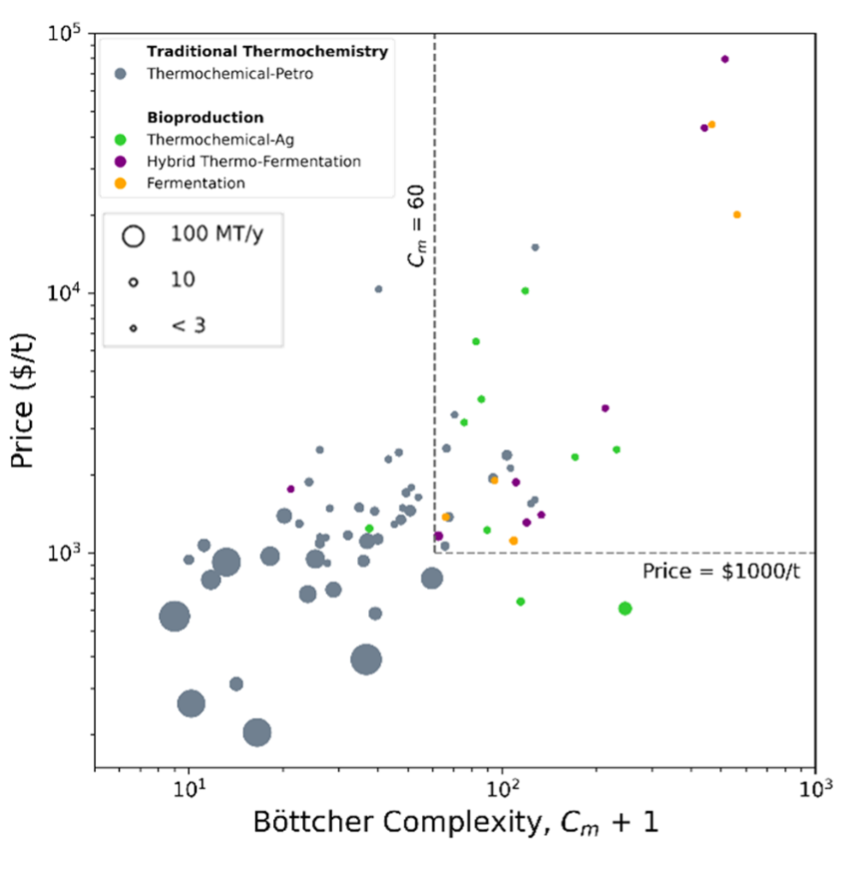
The Role of Synthetic Complexity
Taking these exceptions into account, we considered an alternate metric for complexity based on synthetic complexity, or the total number of chemical conversion steps, N. We count the process steps to convert basic feedstocks such as natural gas, crude oil, oxygen, water, starch, or biomass, into products.[1] For chemicals with multiple production pathways, we considered the most common one.[6] Price vs. N is shown in Figure 2.
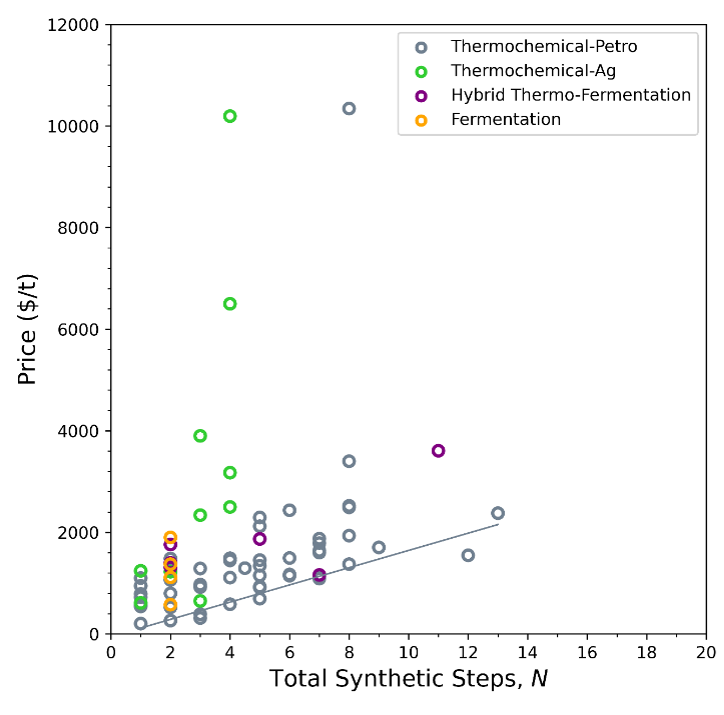
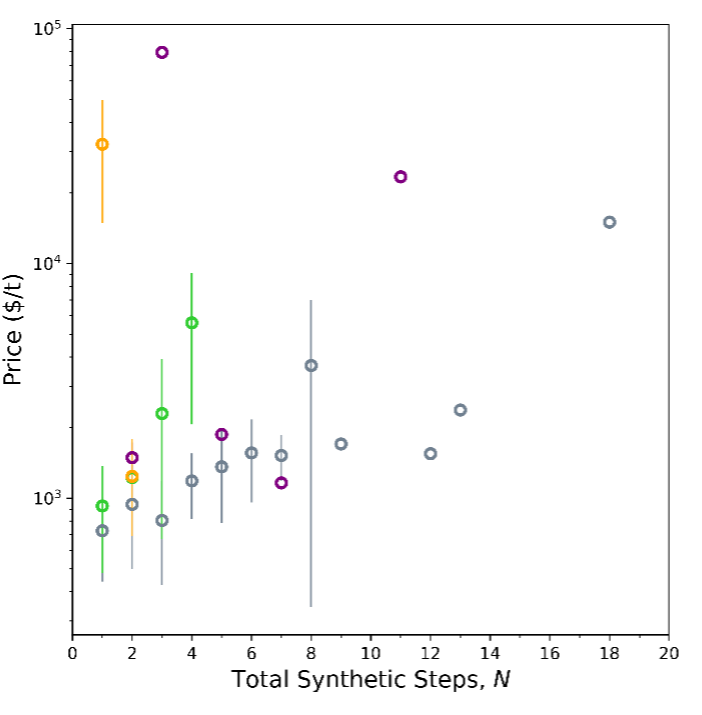
When we plot price and synthetic steps, a few interesting details emerge. As we expect, bioproduction points are more prevalent at low number of steps, but that doesn’t necessarily make them low price. One the other hand, price tends to increase with number of synthetic steps for the Thermochemical-Petro category. The lower bound of this trend gives us an estimate of average minimum cost per step for a thermochemical process: $170/t*step.
We can also compare our two metrics for complexity, Cm, and N (Figure 3) to get a sense of how added synthetic steps contribute to molecular complexity. Bioproduction achieves higher molecular complexity (Cm) in fewer synthetic steps (N), while traditional thermochemistry builds complexity over successive steps. To be precise, for thermochemistry-petro, each step adds an average of 6.4 in molecular complexity. For bioproducts it’s hard to say what the average change in molecular complexity is per step—it varies.
How do Bioproduction and Thermochemistry get used today?
1. Bioproduction is used for chemicals that would take more than 6 thermochemical steps to make.
We can synthesize the information from Figures 2 and 3 to figure out when it makes the most sense to use which process in today’s chemical economy. From Figures 2 and 3, it takes about $170/t to add 6.4 in Cm to a molecule using traditional thermochemistry. Thermochemistry reaches parity with bioproduction, which has a minimum price threshold of $1000/t (Figure 1), in an average of ~6 steps, equivalent to a change in Cm of 38.[1] This implies that for chemical transformations that would take more than 6 thermochemical steps or result in a ΔCm > 38, pursuing a bioprocess route would make a lot of sense.[1] From Figure 1, these conditions tend to occur for molecules with Cm above 60.
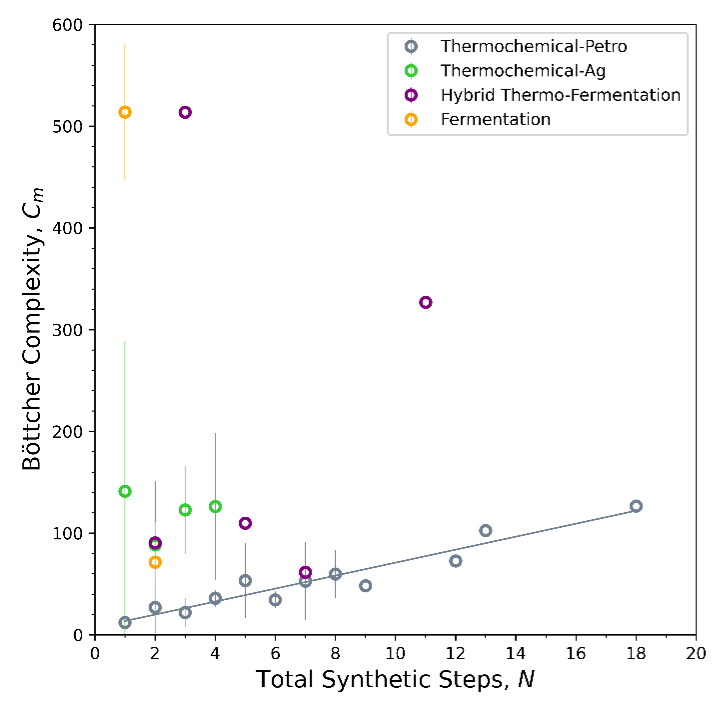
2. Bioproduction is used for reactions that decrease complexity or make large increases in complexity.
We can get a little more specific on how molecules change in thermochemical vs. biological processes if we look at the change in complexity (ΔCm) and price over the final production step (Figure 4).[1] We see a notable division between bioprocesses and thermochemical-petro. In reactions with negative ΔCm, bioproduction is the clear favorite. Thermochemistry is abundant in the region with small positive values of ΔCm, and then bioproduction starts to pick up again at large positive ΔCm. We would see even more bioproduction at large, positive values of ΔCm if we extended the data set went to higher complexities. The first positive ΔCm data point for a fermentative/enzymatic process is at 57 for l-aspartic acid, consistent with our predicted threshold of 38.
Bioproduction gets used in reactions with negative ΔCm for a few reasons. First, it’s partly a consequence of using complex agricultural feedstocks as starting points for these processes. Second, when going from a high complexity starting point to a medium-complexity end point, the finesse of bioprocesses is an asset. Mild conditions and enzyme-directed reactions help prevent unwanted side reactions, which would be hard to prevent if you are indiscriminately dumping energy into a reaction. If your desired endpoint is a very simple molecule like CO2 or CO and you want to smash all the bonds, then the big hammer of thermochemistry makes sense again. This is the case in biomass gasification or combustion
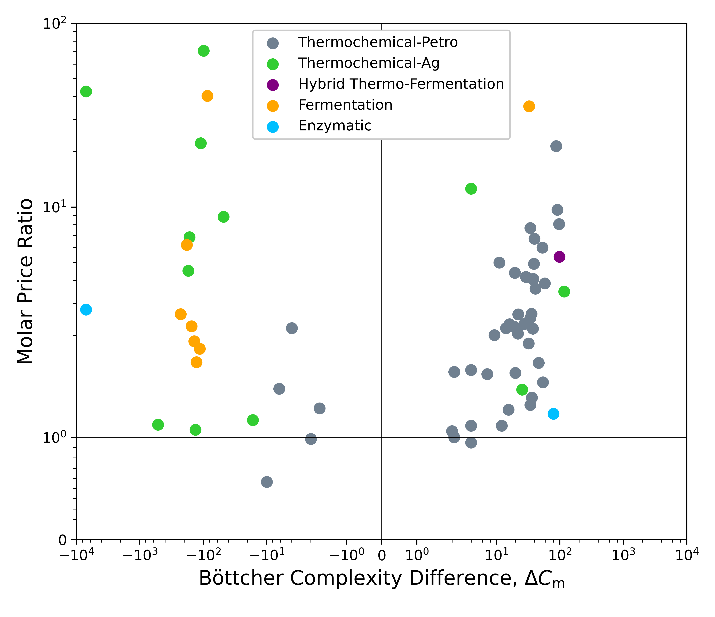
3. Thermochemistry is used when you want cheap embodied energy. Bioproduction is used when you want (relatively) cheap complexity.
Ian’s note pointed out this difference between thermochemistry and bioproduction previously. If you compare the cost per complexity of a molecule to its cost per embodied energy (we use combustion enthalpy for this), bioproduction tends to be used for molecules with cheaper complexity and more expensive embodied energy. Thermochemistry is more often used for molecules with cheap embodied energy (i.e. fuels). See Figure 5. Not too many bioproduction points in the lower right quadrant of the cluster.
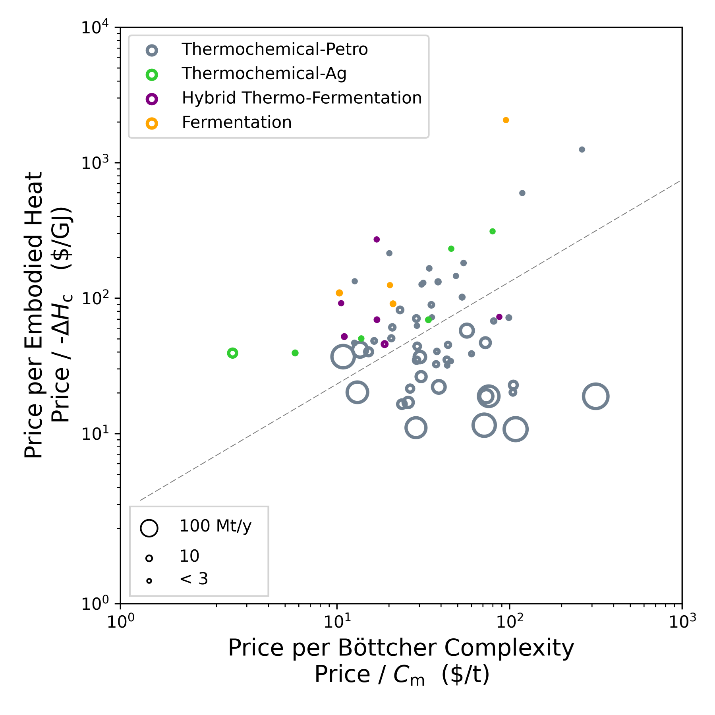
Extrapolating to a Decarbonized Future
When the chemical industry decarbonizes, changes to the complexities and prices of feedstocks will likely shift today’s equilibrium between bioproduction and thermochemistry. Although we don’t expect the boundaries in price and complexity between production methods to completely disappear or flip, bioproduction could become competitive at lower values of Cm than 60.
Such shifts are nothing new. Technology and economic factors are constantly changing. In earlier decades, many thermochemical processes for producing high complexity molecules were replaced by innovations in bioproduction as they matured. For example, the Reichstein process for Vitamin C has been replaced by fermentation of sorbitol. Alternatively, in the 2010s when oil prices were higher, bioproduction projects targeting molecules at complexities below Cm of 60 expanded, for example succinic acid. Many of these efforts collapsed when oil prices later dropped.
Here are two possible changes in a decarbonized future:
1. Decarbonized feedstocks will cost more than our current fossil and purpose-grown agricultural feedstocks.
In the intro, we discussed some of the inherent challenges in using decarbonized feedstocks such as agricultural residues or CO2 reduction products. These challenges could raise the costs of feedstocks, which might shift the boundary between thermochemical and bioproduction to Cm values lower than 60. Let’s assume the cost of bioproduction doesn’t change much in the future. After all, agricultural residues are harder to process, but they are also cheaper than purpose-grown crops. If thermochemical process steps become more expensive than $170/t*step, then bioproduction at $1000/t will become competitive at a smaller number of steps than the current tipping point of N = 6. By our estimate, decarbonized thermochemical feedstocks would have to become ~8.5x more expensive for bioproduction and thermochemistry to compete for 1-step processes.[1] Currently, green H2 is ~4-5x more expensive than dirty H2 from steam methane reforming, lower than this 8.5x factor needed for a complete takeover of bioproduction.
2. Decarbonized feedstocks may require more processing steps.
Decarbonized feedstocks are different from the molecular starting points we have today. Lignocellulosic mixtures found in agricultural residues are more complex than the simple sugars and starches in purpose-grown crops. They require more processing, but they also cost less. On the other hand, synthetic reduction products from CO2 are typically C1 molecules such as CO and formate, whereas fossil feedstocks cover a range of molecular weights including naphtha (up to ~C12) and aromatics. If upgrading decarbonized thermochemical feedstocks requires additional steps, the boundary between thermochemistry and bioproduction would shift to lower Cm by -6.4 for every additional step.
Conclusions
To decarbonize the chemicals industry, we have a choice between the tools of thermochemistry and bioproduction for every product. In the future, we’ll be using decarbonized feedstocks that have different complexities and costs from our current feedstocks. So it is important to understand the role of complexity in chemical manufacturing. Thermochemistry allows you to push reactions far out of equilibrium to maximize productivity, at the cost of high energy input. Bioproduction allows you to access remarkably complex molecules, at the expense of productivity. We’ve assessed the current state of chemical production to see how the advantages and disadvantages of thermochemistry and bioproduction play out today.
Thermochemistry is used for making molecules with a Böttcher complexity index, Cm, below 60 and price below $1000/t. Fossil feedstocks are upgraded over successive steps, at an added complexity of 6.4/step and minimum cost of $170/t*step. Thermochemical reactions are favored for molecules where it’s important to have high embodied energy at low cost. Bioproduction is favored for reactions that involve a negative change in complexity, or a large positive jump in complexity—jumps greater than ΔCm ~ 38. Bioprocesses take place in fewer steps, but not necessarily at low cost.
That’s the state of affairs today, but in the future things will change. If decarbonized thermochemical feedstocks are higher in cost or lower in complexity than fossil feedstocks, bioproduction will be competitive at lower complexities, and for thermochemical processes with fewer reaction steps. Bioproduction will also become more competitive if technology improvements push the price floor below $1000/t or we’re talking about thermochemical products that are above the minimum cost.
We can use the intuition we’ve built up from this analysis to speculate about emerging technologies. Biological nitrogen fixation to make ammonia? Not super likely by this analysis. Companies in this space do not look to compete with thermochemical Haber-Bosch directly on ammonia production. Instead they offer fertilizer compounds with improved performance, and/or better utilization of nitrogen delivered. Gas fermentation to upgrade simple C1 and C2 molecules? Perhaps if the target molecule is sufficiently complex and valuable. Chemical synthesis of sugar? Maybe in environments where access to arable land is restricted—astronaut ice cream anyone? There are reasons to keep at these efforts, but the use cases and scale of adoption might look different from incumbent technologies for the same products.